Christy B. Till1 & Michael Krawczynski2
1School of Earth and Space Exploration, Arizona State University
2Dept. of Earth and Planetary Sciences, Washington University, St. Louis
One of the main techniques we use in our research is experimental petrology. For those who might not be familiar, experimental petrology is the study of geologic materials in the laboratory at simulated pressure and temperature conditions relevant to planetary interiors. High temperature experiments can be conducted in several different apparatus, each specializing in different pressure regimes. Experimental equipment range from those at ambient pressures (typically called “one atmosphere furnaces”) to those using gas/water as a pressure medium (“cold-seal” and “internally heated” pressure vessels) to those using solid pressure medium to attain pressures up to 4 gigapascals (GPa) (“piston cylinders”), up to ~40 GPa (“multi-anvil apparatus”) and ~100-200 GPa (“diamond anvil cells”). Depending on the experimental apparatus and duration of experiment, temperatures accessible in the lab range from 600-2000°C. More detailed explanations of these apparatus can be found here. The modern field of experimental petrology started in the early 1900’s with the construction of high pressure apparatus at Harvard University and high temperature apparatus (as well as the temperature scale) at the Geophysical Lab at the Carnegie Institute in Washington. By the 1960-1970’s the majority of the apparatus designs we use today had been developed.
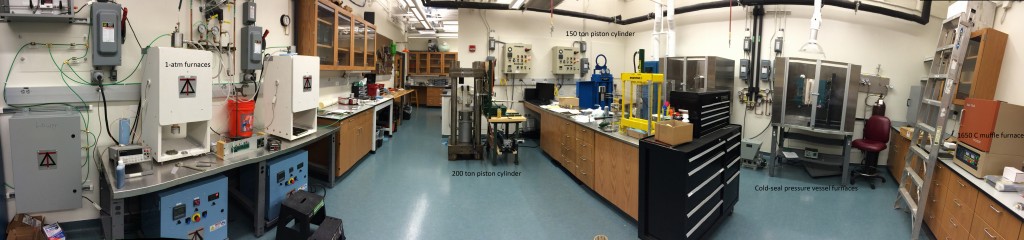
The experimental geochemistry lab in the ESPM group at WUSTL. The lab houses 2 1-atm gas mixing furnaces, 2 piston cylinder devices, and 2 cold-seal pressure vessel furnaces allowing the lab to reach pressures from 1-atm to 35 GPa.
Today, experimental work plays much the same role that it did in the early 20th century; it provides the foundation for making all interpretations of geochemical and isotopic behavior at high temperature in planetary interiors. As technology advances and analytic and theoretical techniques push their boundaries to higher precision, smaller sample sizes, and more exotic element systems, experimental petrology must work along with these studies to provide information on the mechanisms for element transport within planets. Below we describe several exciting research topics where experimental petrology is a key tool, including some of the topics our labs focus on.
Mantle Volatile Storage & Effects on Melting Behavior
One of the scientific advances over the last ten years in which experimental petrology has played a critical role is an understanding of the volatile storage budget of the Earth’s mantle. This includes identifying and quantifying the capacity for nominally anhydrous upper mantle minerals such as olivine, pyroxene and garnet to store and diffuse hydrogen, as well as olivine’s higher pressure polymorphs including wadsleyite and ringwoodite (e.g., Smyth et al., 1991; Panero et al., 2013; Bucholz et al., 2013; Thomas et al., 2015). In addition, large strides have been made in understanding carbon storage in mantle minerals and the deep carbon cycle (e.g., Dasgupta, 2013) and noble gas storage and recycling in the mantle (e.g., Shcheka and Keppler, 2012; Cherniak and Watson 2012; Jackson et al., 2013a). A topic that goes hand-in-hand with storage of volatiles in the mantle is how these volatile concentrations change the melting and rheological behavior of the mantle. Much progress has been made in the last decade regarding our understanding of volatile depression of the mantle solidus, the effect of volatiles on the composition of mantle melts, and the solubility of volatiles in silicate melts. Specifically research has looked at H2O, CO2 and now work is expanding on SO2 and noble gases (e.g., Grove et al., 2006; Dasgupta and Hirschmann, 2010; Till et al., 2012; Schmandt et al., 2014; Green et al., 2014; Fiege et al., 2014; Jackson et al., 2013b).
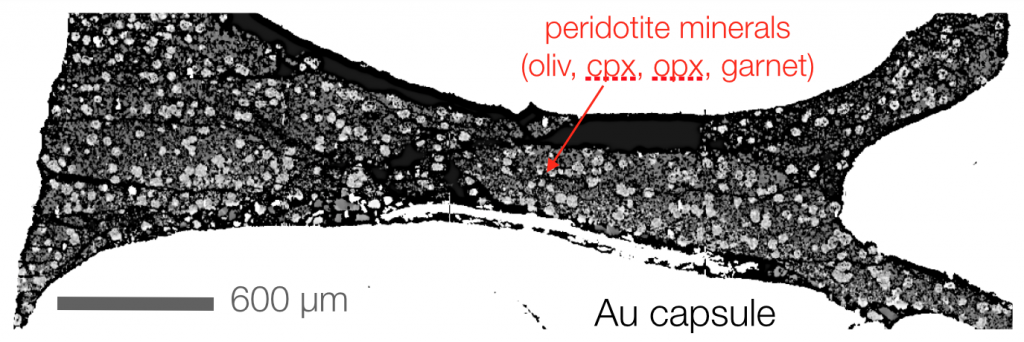
A backscatter electron image of an example piston cylinder experiment (from Till et al., 2012). This experiment was held at 4 GPa for a week, where it grew peridotite minerals from a synthetic oxide mixture of a primitive mantle composition. The white area in the picture is the gold capsule material.
Constraining Diffusivities for Geospeedometry in Magmatic Systems
The technique of geospeedometry is founded on the idea that chemical diffusion of elements and/or isotopes within a crystal, or between a crystal and its matrix, will capture information about the duration of a geologic process as long as the host material has a predictable initial composition that is out of chemical equilibrium with its surroundings. Thus the power of geospeedometry lies in its potential to document rates of processes over a wide range of timescales in any rock that contains chemically zoned minerals. These techniques have been applied to a wide range of geologic problems including the cooling of meteorites (e.g., Yang et al. 2010; Goldstein et al. 2014; Beck et al., 2005; Van Orman et al. 2014), the exhumation of metamorphic provinces (e.g., Lasaga et al., 1977; Ducea et al., 2003; Ganguly et al., 2010) and the tempo of magmatic processes (e.g., Zhang, 1994; Costa et al., 2003; Druitt et al. 2012; Cooper and Kent, 2014;). These studies require experimental investigation into the diffusivities of the elements of interest in the minerals of interest (e.g., Watson and Dohmen, 2010). Diffusivity measurements for elements and isotopes that commonly exhibit zoning in minerals found in mafic to silicic magma compositions is an active area of experimental research (e.g., Cherniak and Watson, 2001; Brady and Cherniak, 2010; Müller et al., 2013; Johnson and Rossman, 2013; Faak et al. 2013; Padrón-Navarta et al., 2014). In addition to diffusion, crystal growth is another kinetic process that can be used to interpret the rates of geologic processes by studying its effects on crystal structure, melt inclusion incorporation and crystal chemistry, for example (e.g., Brugger and Hammer, 2015; Watson and Liang, 1995; Lofgren and Russell, 1986).
Constraints on the Origins of Magmas from Other Planets
Similar to how we are able to understand mantle melting processes by experimentally re-engineering primitive terrestrial basalts, we can also learn something about the melting processes and conditions in other planets by conducting experiments on the composition of volcanic rocks measured on planetary surfaces. Several decades of experimental work on the mare basalts and high-Ti glasses have refined our understanding of the origin of the volcanic and plutonic samples brought back by the Apollo missions (e.g., Elardo et al., 2011; Krawczynski and Grove, 2012). Similar experiments have been conducted to identify the melting scenarios appropriate for Martian meteorites (e.g., Agee and Draper, 2004; Johnson et al., 1991). And now we are able to use analyses from the Martian rovers and other remote sensing missions to collect compositions that can be used for experimental studies (e.g., Monders et al., 2010; Filiberto and Treiman. 2009). For example, measurement of major element ratios by the MESSENGER spacecraft were used to estimate the chemical composition of lavas on the surface of Mercury and experimental investigations of these samples suggest they formed at pressures less <10 kbar near Mercury’s crust-mantle boundary by melting two different mantle lithologies (Charlier et al., 2012; Vander Kaaden et al., 2014). Also experimenters are starting to look outside the solar system, where extra-solar planets may shed light on silicate liquid-vapor equilibrium (e.g., Fegley and Schaefer, 2014) and will likely be relevant to interpret isotopic anomalies in our early solar system (e.g., Richter et al., 2002; Pahlevan et al., 2011).
New Directions All The Time
The field of experimental petrology and geochemistry is constantly pushing new boundaries, collaborating with a wide variety of analysts, using experiments hand in hand with first principles simulations, doing experimental work at synchrotron facilities, and using data collected by remote sensing planetary missions, to name just a few. Other new exciting work includes:
- Experiments combined with molecular dynamics theoretical studies (e.g., Lacks et al., 2012)
- Oxygen fugacity and oxidations mechanisms (e.g., Crabtree and Lange, 2012; Trail et al., 2012; Sharp et al., 2013)
- Trace element behavior during melting (e.g., Matzen et al., 2013)
- Radiogenic isotopic evolution of the mantle (e.g., Bennett and Brenan, 2013)
- Experimental constraints on core formation (e.g., Shahar et al., 2015; Hin et al., 2014)
- Phase transformations at high pressures and temperatures for applications to super-Earths (e.g., McWilliams et al., 2012)
The field is so dynamic we could never hope to capture all of the exciting ongoing science here. We apologize that we only have space to cover a few topics and our list is not meant to be exhaustive or evaluative. To find experimental constraints relevant to your research interests you can visit the Library of Experimental Phase Relations (LEPR) here (Hirschmann et al., 2008). And keep your eyes out at your next scientific meeting to see the many exciting ways in which experimental petrology continues to forward our understanding of solar system geochemistry and beyond!
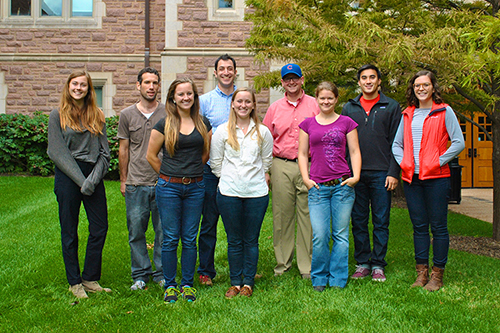
ESPM lab group, from left to right Zoe Lefebvre (UGrad ’16), Yuval Boneh (3rd year Grad), Kelsey Williams (1st year Grad), Phil Skemer, Emily Sexton (UGrad ’15), Mike Krawczynski, Helene Couvy (Research Engineer), Matt Guiang (UGrad ’15), Molly Chaney (UGrad ’16).
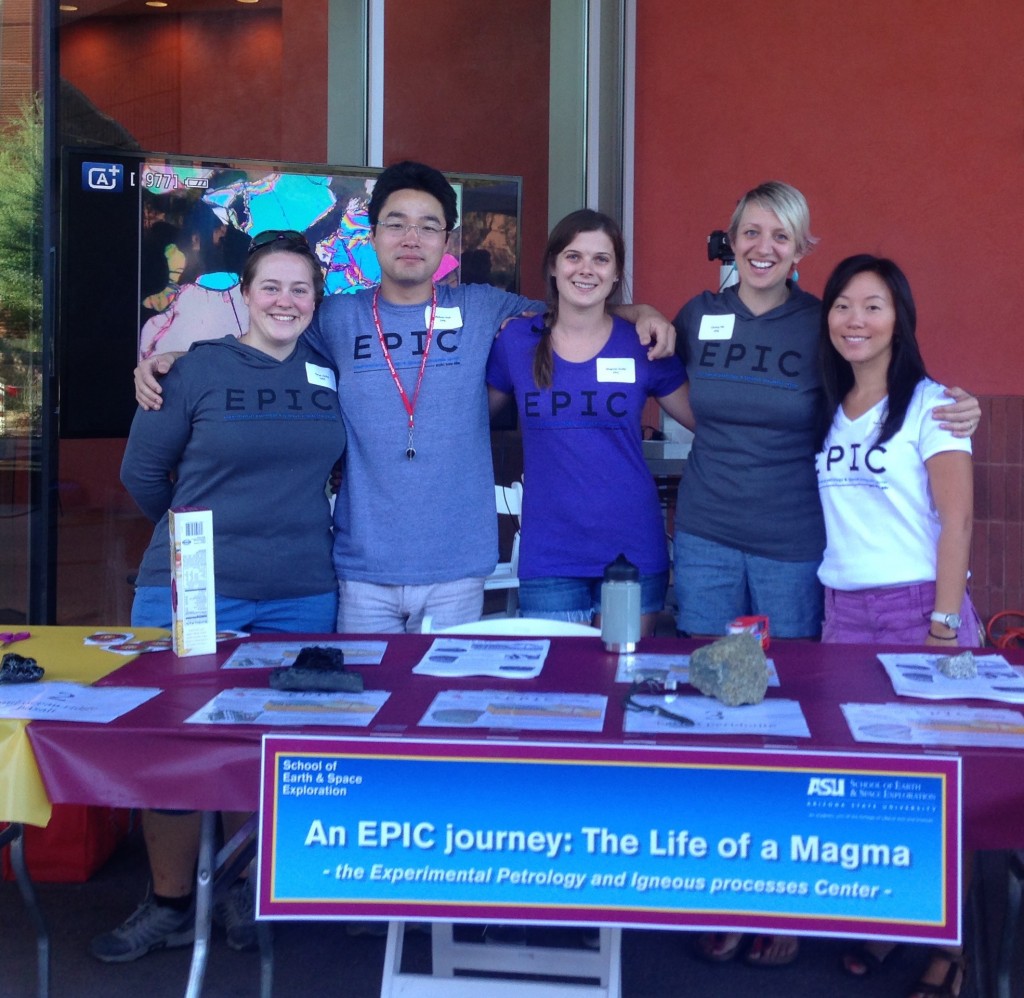
The members of the Experimental Petrology and Igneous processes Center at Arizona State University from left to right: Sarah Cichy (Postdoctoral Fellow), Michael Huh (Laboratory Manager), Meghan Guild (PhD Student), Christy Till (Principal Investigator/Faculty) and Kara Brugman (PhD Student).
References Cited
Agee, C. B., and D. S. Draper (2004), Experimental constraints on the origin of Martian meteorites and the composition of the Martian mantle, Earth and Planetary Science Letters, 224(3-4), 415–429, doi:10.1016/j.epsl.2004.05.022.
Beck, P., M. Chaussidon, J. A. Barrat, P. Gillet, and M. Bohn (2006), Diffusion induced Li isotopic fractionation during the cooling of magmatic rocks: The case of pyroxene phenocrysts from nakhlite meteorites, Geochimica et Cosmochimica Acta, 70(18), 4813–4825, doi:10.1016/j.gca.2006.07.025.
Bennett, N. R., and J. M. Brenan (2013) Controls on the solubility of rhenium in silicate melt: Implications for the osmium isotopic composition of Earth’s mantle, Earth and Planetary Science Letters, 361, 320-332, doi: 10.1016/j.epsl.2012.10.028.
Brady, J. B., and D. J. Cherniak (2010), Diffusion in Minerals: An Overview of Published Experimental Diffusion Data, Reviews in Mineralogy and Geochemistry, 72(1), 899–920, doi:10.2138/rmg.2010.72.20.
Brugger, C. R., and J. E. Hammer (2015), Prevalence of growth twins among anhedral plagioclase microlites, American Mineralogist, 100(2-3), 385–395, doi:10.2138/am-2015-4809.
Bucholz, C. E., G. A. Gaetani, M. D. Behn, and N. Shimizu (2013), Earth and Planetary Science Letters, Earth and Planetary Science Letters, 374(C), 145–155, doi:10.1016/j.epsl.2013.05.033.
Charlier, B., T. L. Grove, and M. T. Zuber (2013), Earth and Planetary Science Letters, Earth and Planetary Science Letters, 363(C), 50–60, doi:10.1016/j.epsl.2012.12.021.
Cherniak, D. J., and E. B. Watson (2001), Pb diffusion in zircon, Chemical Geology, 172(1-2), 5–24, doi:10.1016/S0009-2541(00)00421-6.
Cooper, K. M., and A. J. R. Kent (2014), Rapid remobilization of magmatic crystals kept in cold storage, Nature, 506, 480–483, doi:10.1038/nature12991.
Costa, F., S. Chakraborty, and R. Dohmen (2003), Diffusion coupling between trace and major elements and a model for calculation of magma residence times using plagioclase, Geochimica et Cosmochimica Acta, 67(12), 2189–2200, doi:10.1016/S0016-7037(02)01345-5.
Crabtree, S. M., and R. A. Lange (2011), An evaluation of the effect of degassing on the oxidation state of hydrous andesite and dacite magmas: a comparison of pre- and post-eruptive Fe2+ concentrations, Contrib Mineral Petrol, 163(2), 209–224, doi:10.1007/s00410-011-0667-7.
Dasgupta, R. (2013), Ingassing, Storage, and Outgassing of Terrestrial Carbon through Geologic Time, Reviews in Mineralogy and Geochemistry, 75(1), 183–229, doi:10.2138/rmg.2013.75.7.
Dasgupta, R., and M. M. Hirschmann (2010), The deep carbon cycle and melting in Earth’s interior, Earth and Planetary Science Letters, 298(1-2), 1–13, doi:10.1016/j.epsl.2010.06.039.
Druitt, T. H., F. Costa, E. Deloule, M. Dungan, and B. Scaillet (2012), Decadal to monthly timescales of magma transfer and reservoir growth at a caldera volcano, Nature, 482(7383), 77–80, doi:10.1038/nature10706.
Ducea, M. N., J. Ganguly, E. J. Rosenberg, P. J. Patchett, W. J. Cheng, and C. Isachsen (2003), Sm-Nd dating of spatially controlled domains of garnet single crystals: a new method of high-temperature thermochronology, Earth and Planetary Science Letters, 213(1-2), 31–42, doi:10.1016/S0012-821X(03)00298-X.
Elardo, S. M., D. S. Draper, and C. K. Shearer Jr. (2011) Lunar Magma Ocean crystallization revisted: Bulk composition, early cumulate mineralogy, and the source regions of the highlands Mg-suite, Geochimica et Cosmochimica Acta, 75, 3024-3045, doi:10.1016/j.gca.2011.02.033.
Faak, A., S. Chakraborty, L. A. Coogan (2013) Mg in plagioclase: Experimental calibration of a new geothermometer and diffusion coefficients, Geochimica et Cosmochimica Acta, 123, 195-217, doi:10.1016/j.gca.2013.05.009.
Fegley Jr., B., and L. K. Schaefer (2014) 6.3 Chemistry of Earth’s Earliest Atmosphere, in: Treatise on Geochemistry 2nd Edition, p. 71-90, doi: 10.1016/B978-0-08-095975-7.01303-6.
Fiege, A., F. Holtz, N. Shimizu, C. W. Mandeville, H. Behrens, and J. L. Knipping (2014), Sulfur isotope fractionation between fluid and andesitic melt: An experimental study, Geochimica et Cosmochimica Acta, 142(C), 501–521, doi:10.1016/j.gca.2014.07.015.
Filiberto, J., and A. H. Treiman (2009) The effect of chlorine on the liquidus of basalt: First results and implications for basalt genesis on Mars and Earth, Chemical Geology, 264 (1-4), 60-68, doi: 10.1016/j.chemgeo.2008.08.025.
Ganguly, J. (2010), Cation Diffusion Kinetics in Aluminosilicate Garnets and Geological Applications, Reviews in Mineralogy and Geochemistry, 72(1), 559–601, doi:10.2138/rmg.2010.72.12.
Goldstein, J. I., J. Yang, and E. R. D. Scott (2014) Determining cooling rates of iron and stony-iron meteorites from measurements of Ni and Co at kamacite-taenite interfaces, Geochimica et Cosmochimica Acta, 140, 297-320, doi:10.1016/j.gca.2014.05.025.
Green, D. H., W. O. Hibberson, A. Rosenthal, I. Kovacs, G. M. Yaxley, T. J. Falloon, and F. Brink (2014), Experimental Study of the Influence of Water on Melting and Phase Assemblages in the Upper Mantle, Journal of Petrology, 55(10), 2067–2096, doi:10.1093/petrology/egu050.
Grove, T. L., and M. J. Krawczynski (2009), Lunar Mare Volcanism: Where Did the Magmas Come From? Elements, 5(1), 29–34, doi:10.2113/gselements.5.1.29.
Grove, T., N. Chatterjee, S. Parman, and E. Médard (2006), The influence of H2O on mantle wedge melting, Earth and Planetary Science Letters, 249(1-2), 74–89, doi:10.1016/j.epsl.2006.06.043.
Hin, R. C., C. Fitoussi, M. W. Schmidt, and B. Bourdon (2014) Experimental determination of the Si isotope fractionation factor between liquid metal and liquid silicate, Earth and Planetary Science Letters, 387, 55-66, doi: 10.1016/j.epsl.2013.11.016.
Hirschmann, M. M., M. S. Ghiorso, F. A. Davis, S. M. Gordon, S. Mukherjee, T. L. Grove, M. Krawczynski, E. Médard, and C. B. Till (2008), Library of Experimental Phase Relations (LEPR): A database and Web portal for experimental magmatic phase equilibria data, Geochem. Geophys. Geosyst., 9(3), n/a–n/a, doi:10.1029/2007GC001894.
Jackson, C. R. M., S. W. Parman, S. P. Kelley, and R. F. Cooper (2013a), Constraints on light noble gas partitioning at the conditions of spinel-peridotite melting, Earth and Planetary Science Letters, 384(C), 178–187, doi:10.1016/j.epsl.2013.09.046.
Jackson, C. R. M., S. W. Parman, S. P. Kelley, and R. F. Cooper (2013b), Noble gas transport into the mantle facilitated by high solubility in amphibole, Nature Publishing Group, 6(7), 562–565, doi:10.1038/ngeo1851.
Johnson, E. A., and G. R. Rossman (2013), The diffusion behavior of hydrogen in plagioclase feldspar at 800-1000 C: Implications for re-equilibration of hydroxyl in volcanic phenocrysts, American Mineralogist, 98(10), 1779–1787, doi:10.2138/am.2013.4521.
Johnson, M. C., M. J. Rutherford, and P. C. Hess (1991) Chassigny petrogenesis: Melt compositions, intensive parameters and water contents of Martian (?) magmas, Geochimica et Cosmochimica Acta, 55, 349-366, doi:10.1016/0016-7037(91)90423-3.
Krawczynski, M.J., and Grove, T.L. (2012) Experimental investigation of the influence of oxygen fugacity on the source depths for high titanium lunar ultramafic magmas, Geochimica et Cosmochimica Acta, 79, 1–19, doi: 10.1016/j.gca.2011.10.043.
Lacks, D. J., G. Goel, C. J. Bopp IV, J. A. Van Orman, C. E. Lesher, and C. C. Lundstrom (2012) Isotope Fractionation by Thermal Diffusion in Silicate Melts, Physical Review Letters, 108, 065901, doi: 10.1013/PhysRevLett.108.065901.
Lasaga, A. C., S. M. Richardson, and H. D. Holland (1977), The Mathematics of Cation Diffusion and Exchange Between Silicate Minerals During Retrograde Metamorphism, in Energetics of Geological Processes, pp. 353–388, Springer Berlin Heidelberg, Berlin, Heidelberg.
Lofgren, G. and W. J. Russell (1986) Dynamic crystallization of chondrule melts of porphyritic and radial pyroxene composition, Geochimica et Cosmochimica Acta, 50, 1715-1726, doi:10.1016/0016-7037(86)90133-X.
Matzen, A.K., Baker, M.B., Beckett, J.R., and Stolper, E.M. (2013) The Temperature and Pressure Dependence of Nickel Partitioning between Olivine and Silicate Melt, Journal of Petrology, 54, 2521–2545, doi: 10.1093/petrology/egt055.
McWilliams, R. S., D. K. Spaulding, J. H. Eggert, and P. M. Celliers (2012), Phase transformations and metallization of magnesium oxide at high pressure and temperature, Science, doi:10.1016/j.cattod.2012.06.001.
Monders, A. G., E. Médard, and T. L. Grove (2007), Phase equilibrium investigations of the Adirondack class basalts from the Gusev plains, Gusev crater, Mars, Meteoritics & Planetary Science, 42(1), 131–148, doi:10.1111/j.1945-5100.2007.tb00222.x.
Mueller, T., R. Dohmen, H. W. Becker, J. H. ter Heege, and S. Chakraborty (2013), Fe-Mg interdiffusion rates in clinopyroxene: experimental data and implications for Fe-Mg exchange geothermometers, Contrib Mineral Petrol, 166(6), 1563–1576, doi:10.1007/s00410-013-0941-y.
Padrón-Navarta, J. A., J. Hermann, and H. S. C. O’Neill (2014), Site-specific hydrogen diffusion rates in forsterite, Earth and Planetary Science Letters, 392(C), 100–112, doi:10.1016/j.epsl.2014.01.055.
Pahlevan K., D. J. Stevenson, and J. M. Eiler (2011) Chemical fractionation in the silicate vapor atmosphere of the Earth, Earth and Planetary Science Letters, 301(3-4), 433-443, doi:10.1016/j.epsl.2010.10.036.
Panero, W. R., J. R. Smyth, J. S. Pigott, Z. Liu, and D. J. Frost (2013), Hydrous ringwoodite to 5 K and 35 GPa: Multiple hydrogen bonding sites resolved with FTIR spectroscopy, American Mineralogist, 98(4), 637–642, doi:10.2138/am.2013.3978.
Richter, F. M., A. M. Davis, D. S. Ebel, and A. Hashimoto (2002) Elemental and isotopic fractionation of Type B calcium-, aluminum-rich inclusions: experiments, theoretical considerations, and constraints on their thermal evolution, Geochimica et Cosmochimica Acta, 66, 521-540, doi:10.1016/S0016-7037(01)00782-7.
Schmandt, B., S. D. Jacobsen, T. W. Becker, and Z. Liu (2014), Dehydration melting at the top of the lower mantle, Science, doi:10.1126/science.1253258.
Shahar, A., V. J. Hillgren, M. F. Horan, J. Mesa-Garcia, L. A. Kaufman, and T.D. Mock (2015) Sulfur-controlled iron isotope fractionation experiments of core formation in planetary bodies, Geochimica et Cosmochimica Acta, 150, 253-264, doi:10.1016/j.gca.2014.08.011.
Sharp, Z.D., McCubbin, F.M., and Shearer, C.K. (2013) A hydrogen-based oxidation mechanism relevant to planetary formation, Earth and Planetary Science Letters, 380, 88–97, doi: 10.1016/j.epsl.2013.08.015.
Shcheka, S. S., and H. Keppler (2012), The origin of the terrestrial noble-gas signature, Nature, 490(7421), 531–534, doi:10.1038/nature11506.
Smyth, J. R., D. R. Bell, and G. R. Rossman (1991), Incorporation of Hydroxyl in Upper-Mantle Clinopyroxenes, Nature, 351(6329), 732–735, doi:10.1038/351732a0.
Thomas, S.-M., S. D. Jacobsen, C. R. Bina, P. Reichart, M. Moser, E. H. Hauri, M. Koch-MÃ ller, J. R. Smyth, and G. Dollinger (2015), Quantification of water in hydrous ringwoodite, frontiers in Earth Science, 2, 1–10, doi:10.3389/feart.2014.00038.
Till, C.B., Grove, T., and Withers, A.C. (2012) The beginnings of hydrous mantle wedge melting, Contributions to Mineralogy and Petrology, 163, 669–688, doi: 10.1007/s00410-011-0692-6.
Trail, D., E. B. Watson, and N. D. Tailby (2012), Ce and Eu anomalies in zircon as proxies for the oxidation state of magmas, Geochimica et Cosmochimica Acta, 97(C), 70–87, doi:10.1016/j.gca.2012.08.032.
Van Orman, J. A., D. J. Cherniak, and N. T. Kita (2014), Magnesium diffusion in plagioclase: Dependence on composition, and implications for thermal resetting of the 26Al–26Mg early solar system chronometer, Earth and Planetary Science Letters, 385(C), 79–88, doi:10.1016/j.epsl.2013.10.026.
Vander Kaaden, K. E., F. M. McCubbin, and A. S. Bell (2014) Phase Equilibrium Experiments on Analogue Lavas from the Northern Volcanic Plains of Mercury: Implications for the Mineralogy of the Mercurian Mantle, Lunar and Planetary Science Conference (45), Abstract #1906.
Watson, E. B., and R. Dohmen (2010), Non-traditional and Emerging Methods for Characterizing Diffusion in Minerals and Mineral Aggregates, Reviews in Mineralogy and Geochemistry, 72(1), 61–105, doi:10.2138/rmg.2010.72.3.
Watson, E. B., and Y. Liang (1995) A simple model for sector zoning in slowly grown crystals: Implications for growth rate and lattice diffusion, with emphasis on accessory minerals in crustal rocks, American Mineralogist, 80, 1179-1187.
Yang, J., J. I. Goldstein, and E. R. D. Scott (2010) Main-group pallasites: Thermal history, relationship to IIIAB irons, and origin, Geochimica et Cosmochimica Acta, 74, 4471-4492, doi:10.1016/j.gca.2010.04.016.
Zhang, Y. (1994), Reaction kinetics, geospeedometry, and relaxation theory, Earth and Planetary Science Letters, 122(3-4), 373–391, doi:10.1016/0012-821X(94)90009-4.